Relevance
Background
Building structures at the nanoscale can prove challenging as many forces and effects that are inconsequential at larger scales become highly significant. Considerations such as Brownian motion, viscosity, susceptibility to intermolecular forces and even weak interactions can make nanoscale assembly near impossible. With the development of a new technique called DNA origami, programmable self-assembly of distinct DNA structures is possible [1]. Since its invention, DNA origami nanostructures have progressed from simple 2D shapes to functional 3D nanomachines [2].
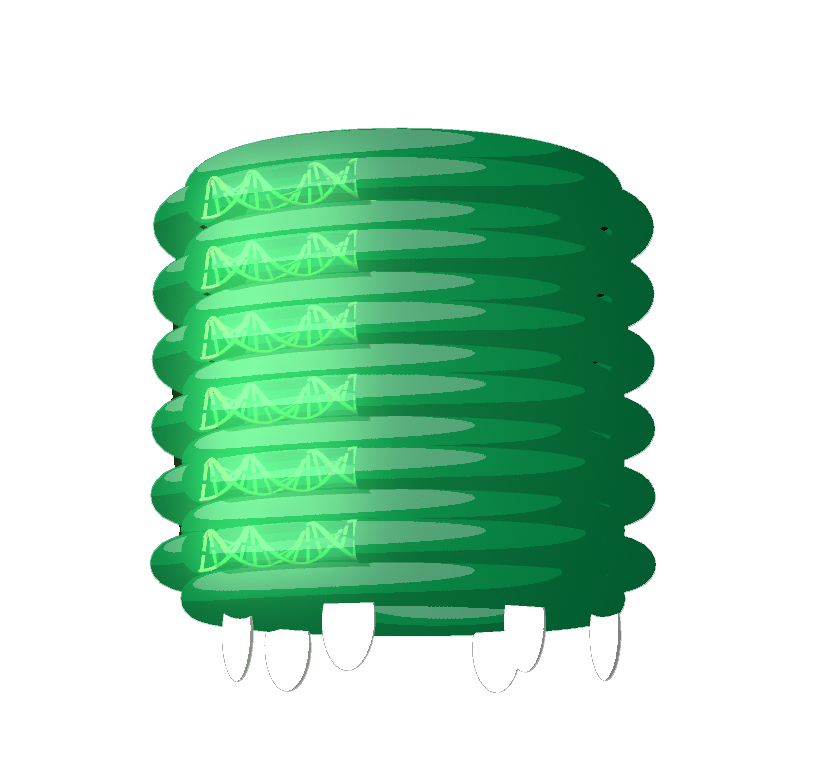
A hot area of research in this field is DNA origami nanostructures that are involved in lipid interactions. By incorporating hydrophobic molecules like cholesterol and porphyrin to DNA origami nanostructures they can embed in lipid membranes [3-6]. A range of lipid-interacting DNA nanopores have been reported with functions from mimicking biological ion channels by induction of ionic currents to scrambling and perturbing biological membranes and potentially inducing cell death [4,5]. These are all exciting functional nanostructures that could potentially be significant to research in biochemistry and the development of clinically useful novel therapeutics. However, a switchable origami where lipid interactions can be controlled is yet to be constructed.
The problem
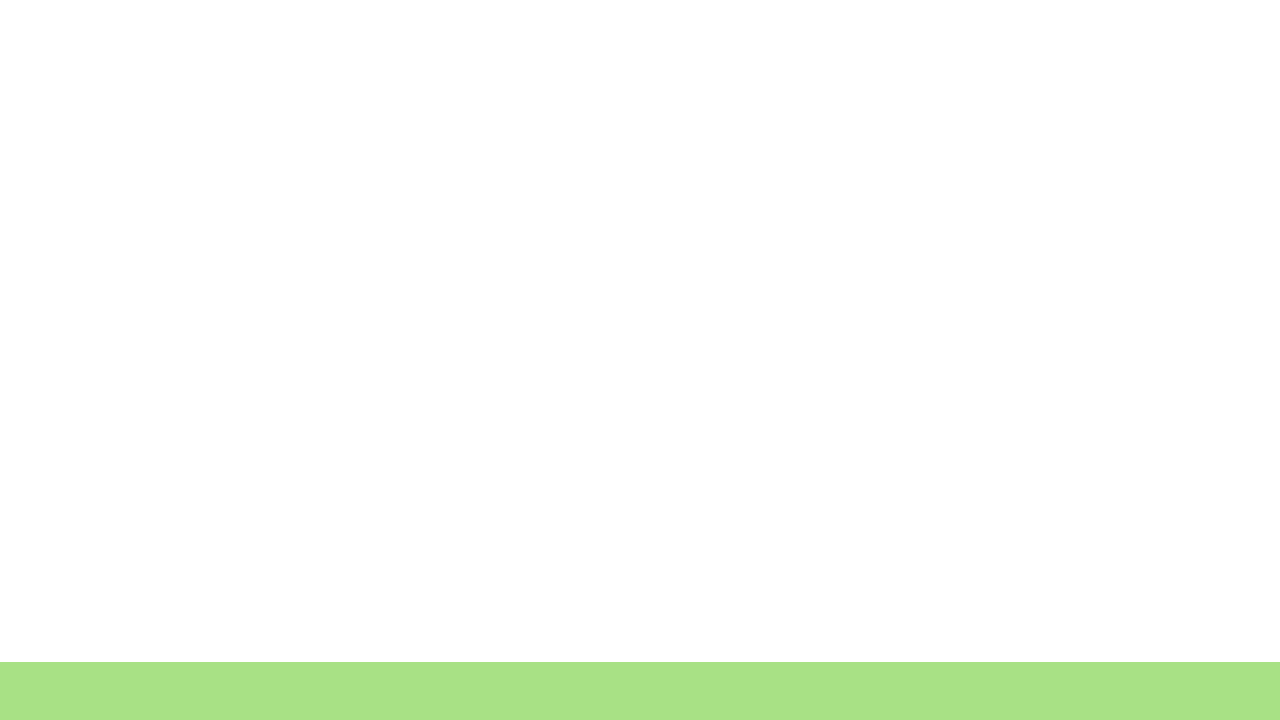
For our BIOMOD project we chose to tackle a highly topical issue; the threat posed by widespread antimicrobial resistance. Antimicrobial resistance occurs when harmful microorganisms; bacteria, viruses, fungi and protozoa, evolve to grow in the presence of medications that in the past would normally have killed or stopped their growth. This has led to the emergence of ‘superbugs’ such as Staphylococcus aureus. Antimicrobial resistance is a significant global health issue leading to rising costs in healthcare. As traditional treatments become ineffective, longer hospitalisations and more expensive drugs are required. In fact drug resistant infections are implicated in nearly 700 000 deaths yearly [7]. In a recent study commissioned by the UK government, it was predicted that by 2050, 10 million people will die from antimicrobial resistance each year without significant interventions on this issue [7]. To put that into perspective, the number of deaths from drug resistant infections will surpass that of deaths currently caused by cancer [8]. Thus, the demand for novel therapeutics with entirely distinct mechanisms of action from current therapeutics are needed to address such a pressing issue.
The enemy - bacteria:
Bacterial cell walls are highly complex and display a wide range of variation between genera and species. They are categorised as Gram-positive or Gram-negative depending on the result that they give when assayed in a Gram-stain test (external link). This result gives information about the make-up of the bacterial cell wall.
We recognise the intricacy of bacterial cell walls which differ between gram positive and gram negative bacteria in contrast to mammalian cell membranes. Unlike other organisms, the bacterial cell wall is made up of a polymer called peptidoglycan, which consists of sugars and amino acids and gives structural strength to the bacteria. Gram-positive bacteria have a thick peptidoglycan layer in their cell wall surrounding their plasma membrane, whereas gram-negative bacteria have a peptidoglycan layer sandwiched between an inner and outer membrane.
However, we can start by focusing on the plasma/cytoplasmic membrane (phospholipid bilayer) of bacterial cells. Liposomes can be used to simulate this component of the cell wall. Although these spherical-shaped vesicles are uniform and do not have the complex lipid organisation known to exist in biological systems, such as lipid rafts, they allow for hydrophobic moieties to embed in the phospholipid bilayer. Therefore, for proof of concept studies liposomes can act as a simplified model for bacterial cells.
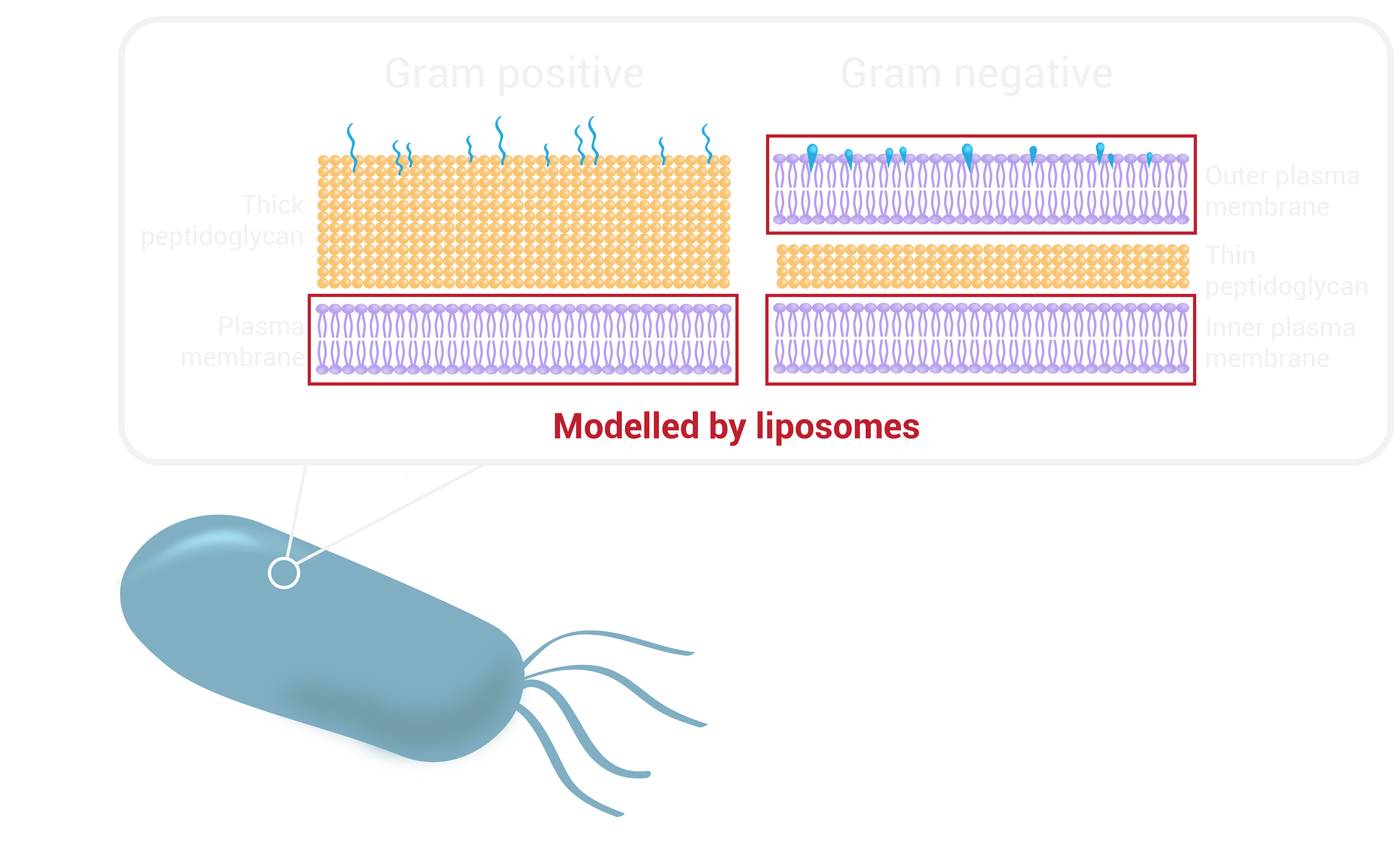
Merit
Our solution
In nature, antimicrobial peptides occur naturally and are produced by organisms as a first-line of defence. They act by destabilising biological membranes [9]. These antimicrobial peptides have been reported to create pores that result in leakage of cellular components [10]. We were inspired by nature’s defence system and the use of pores for destruction of microbes in combination with existing work in the field on DNA nanopores. The aim of our project is to design a switchable DNA nanopore that can be used to combat antimicrobial resistance by selectively punching holes and disrupting homeostasis in harmful bacteria. This approach is different to traditional chemical drug-based approaches and offers a novel mechanism of action to potentially tackle antimicrobial resistance. Our nanopore system could also be used alongside existing antibiotics to increase their efficacy, by making holes through which antibiotics are able to enter the bacteria more easily.
Our nanopore is decorated with cholesterol moieties to allow it to embed into the bacterial membranes to create a physical hole for molecules to flow through. It is anticipated that this pore will disrupt biological membranes and the internal ionic concentration of the bacteria, resulting in bacterial death.
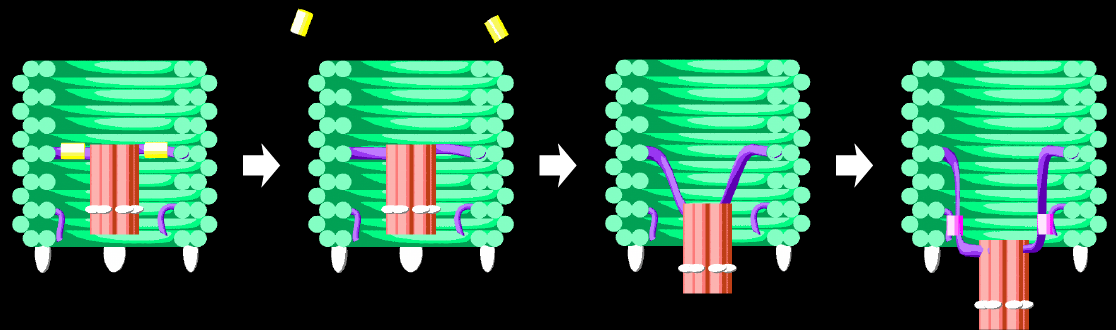
However, the nanopore insertion is mediated by non-specific hydrophobic interactions between cholesterols and all lipid membranes. As a result, no selectivity can be achieved between human cells, healthy bacteria and harmful bacteria. To achieve selectivity, our nanopore will be enclosed in a DNA barrel and will only be released when it reaches a specific target. In its ‘switched-off’ state the nanopore will be held tightly inside the barrel such that the nanopore is not free to insert into lipid membranes. Once switched on, the nanopore will be free to move and access a range of conformations via Brownian motion. Once the nanopore finds it way out of the barrel it is free to insert into lipid membranes resulting in destruction of the target. To ensure that the nanopore remains out of the barrel once released, it will be locked open to maximise nanopore-membrane interactions.
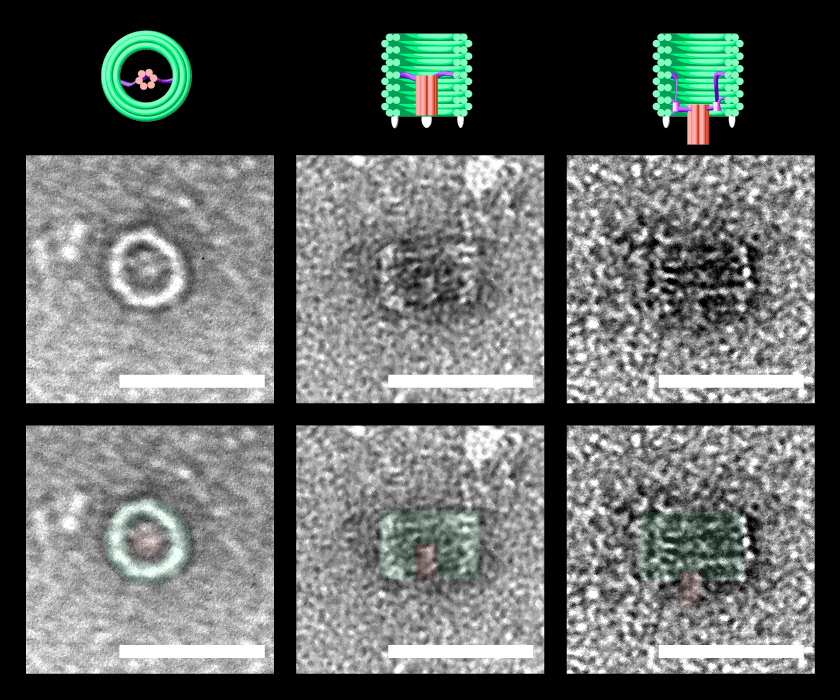
TEM images of nanoscale DNAlien - showing activation for bacterial destruction. The pore is hidden inside the barrel in the inactive state (top view - left panel, side view - middle panel). When activated, the pore can move outside the barrel to perforate bacterial membranes, and destroy the bacteria (side view - right panel). 3D Models (top), TEM images (middle), and false colour annotated TEM images (bottom) are all shown to scale. Scale bars = 50 nm.
The switch mechanism will involve a cocktail of aptamers specific to a microbial target, which are attached to the switchable DNA nanopore. Aptamers are single-stranded DNA/RNA molecules, with the ability to bind specific molecular targets with high affinity. As there are six different attachment sites on the DNA barrel concealing the nanopore, numerous cocktail combinations of aptamers will be tested to enhance and determine the greatest sensing signal for the detection of bacteria [11]. This will guide the nanopore to the bacteria, allowing for the differentiation of harmful bacterial cells from healthy human cells, and beneficial bacteria. Upon binding of the DNA barrel to the microbe, the nanopore will be released to penetrate the microbial lipid membrane beneath for destruction, achieving selective destruction.
To execute this plan of attack, bacterial targets with known aptamers will be tested for binding of the switchable DNA nanopore to the microbe. Out initial focus will be on Escherichia coli, which causes gastrointestinal problems, and Staphylococcus aureus, which can become highly resistant and potentially fatal. Aptamers that have a high specificity and affinity for the respective targets have been developed for strains of both E. coli [11] and S. aureus [12], as well as Mycobacterium tuberculosis, which has infected one-third of the world’s population [13]. As non-pathogenic lab strains of E. coli are widely studied, this bacteria will be the first test case for targeting experiments.
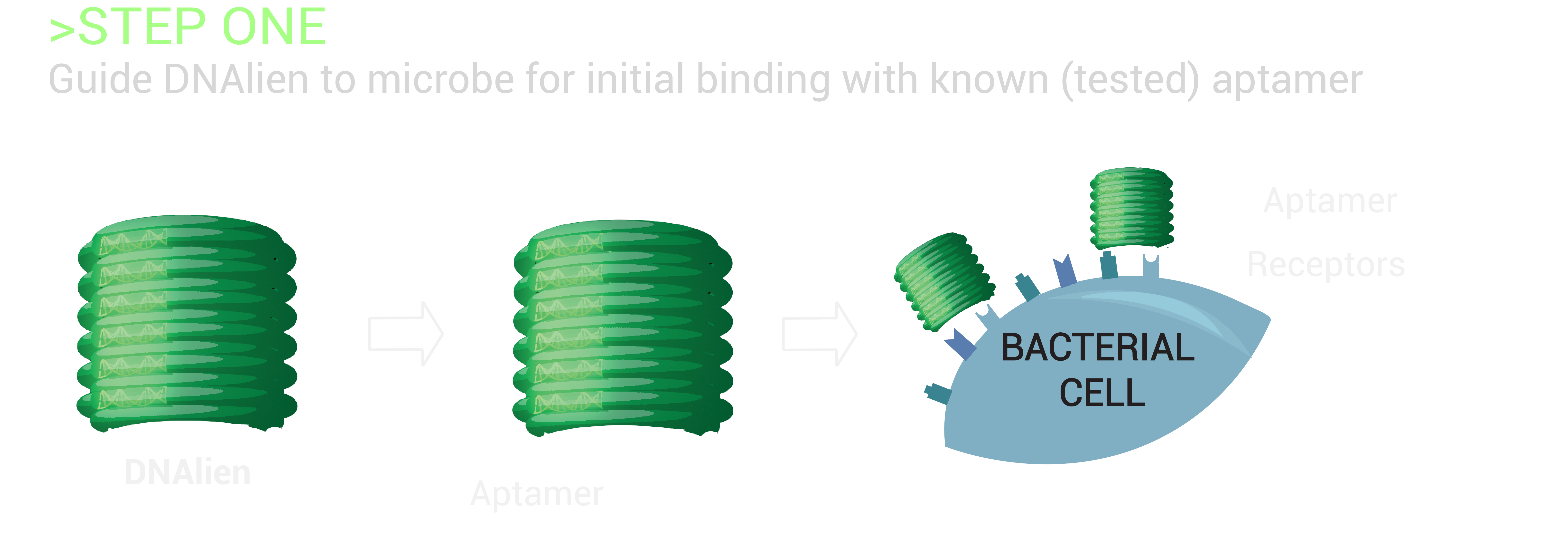
To further enhance binding capacity and achieve greater selectivity of targeting specific microbes, additional bacterial markers will be investigated. Possible target molecules to be considered include Outer Membrane Protein A (OmpA) of E. coli, as this protein is embedded in the outer membrane and more easily accessible [15], as well as collagen like proteins found to be present in thin hair-like surface filaments of other bacteria [16]. These bacterial proteins will be targeted by synthesising new aptamers using Systematic Evolution of Ligands by Exponential Enrichment (SELEX) [13-14]. SELEX is a combinatorial chemistry technique, which can be used to evolve aptamers through multiple rounds of in vitro selection, to achieve extremely high binding affinity and target selectivity to a variety of target ligands.
These additional aptamers will be conjugated to the remaining attachment sites on the DNA barrel, thereby creating a cocktail of aptamers. By testing many possible combinations, we will determine the optimal set of aptamers for targeting of specific strains of resistant bacteria.
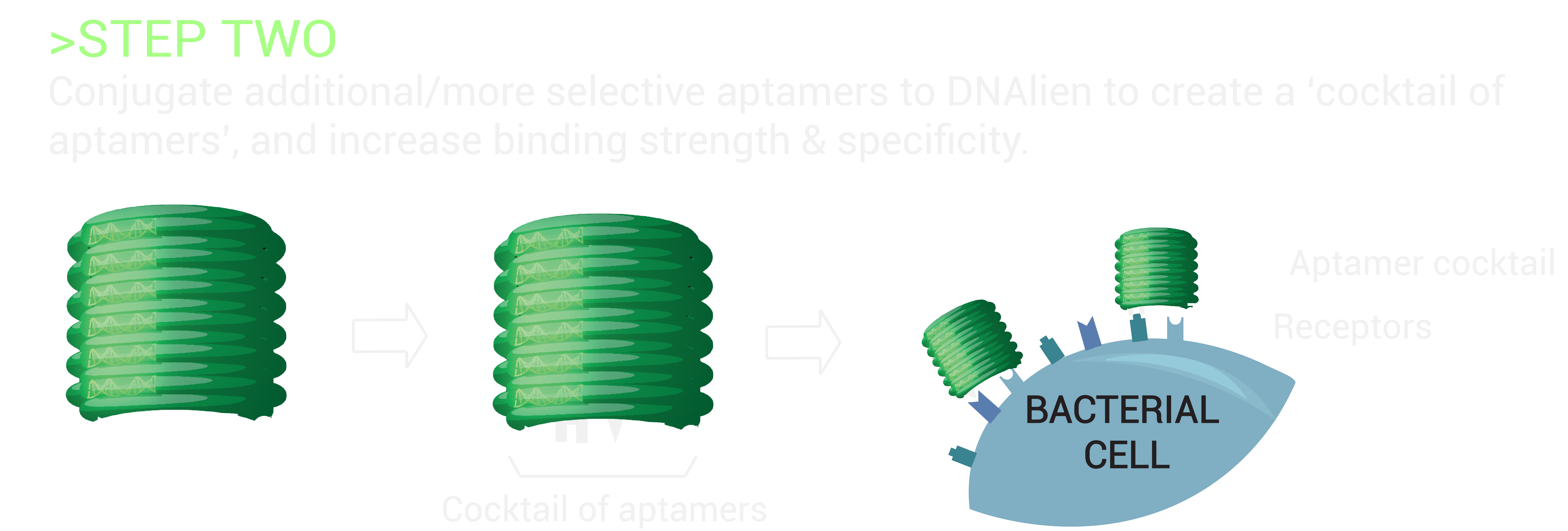
Specification
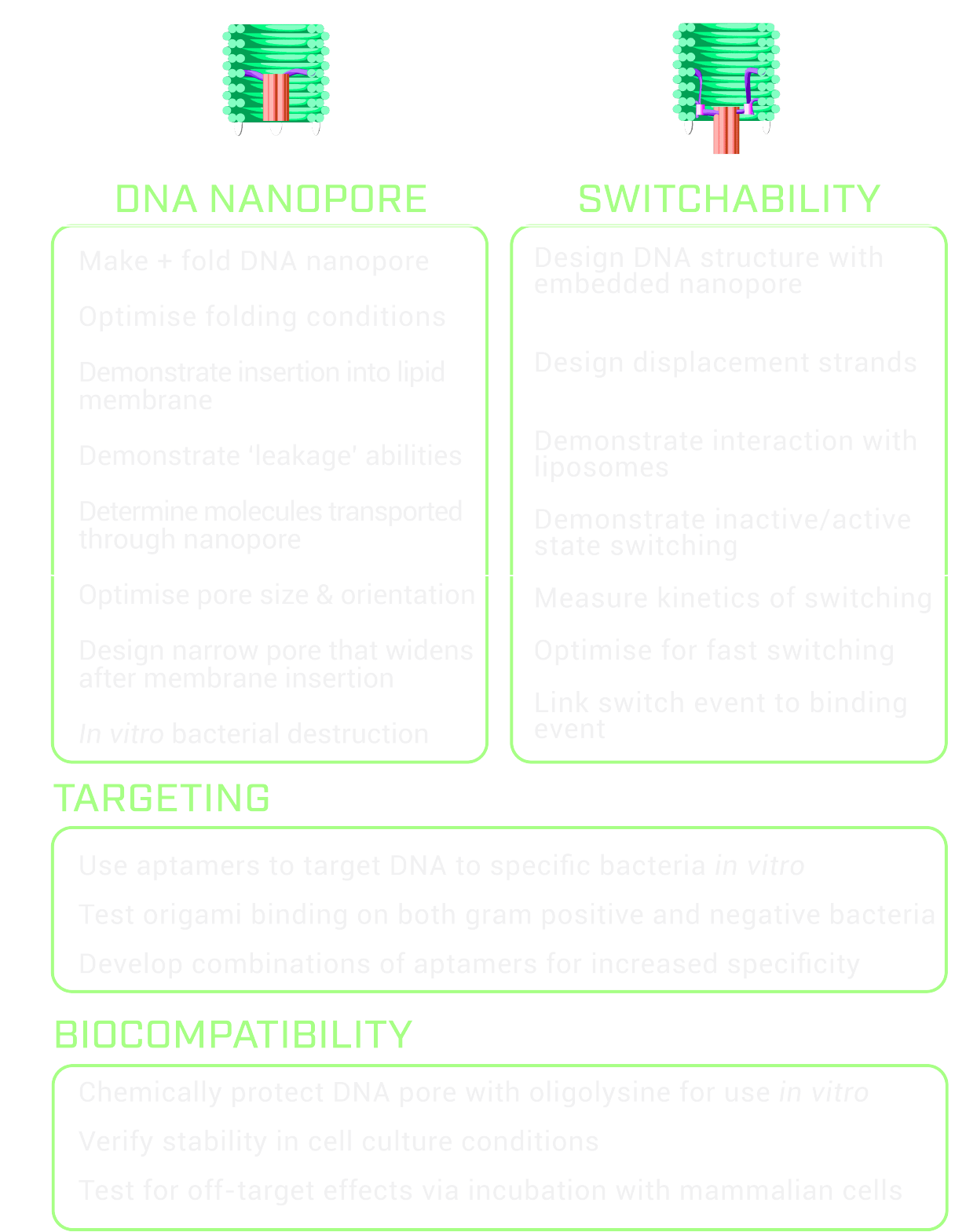
Project goals
The ultimate goal of this project is to design a switchable DNA nanopore for the selective destruction of microbes. Our project can be divided into two distinct sections; targeting and destruction.
Given the timeframe of BIOMOD, we decided to focus our research on the destruction aspect of the project and and will test the specific targeting of microbes in future work. Our goal was to design a DNA nanopore that can embed into lipid membranes and to design a switch mechanism to control this membrane insertion.
As a proof of concept, we decided to use DNA displacement strands as a simplification of the trigger for the on/off state of the origami in an attempt to demonstrate that the DNA origami can be switched between two states. An autonomous trigger, driven by aptamer binding to the bacteria, will be developed in future work.
Criteria for success
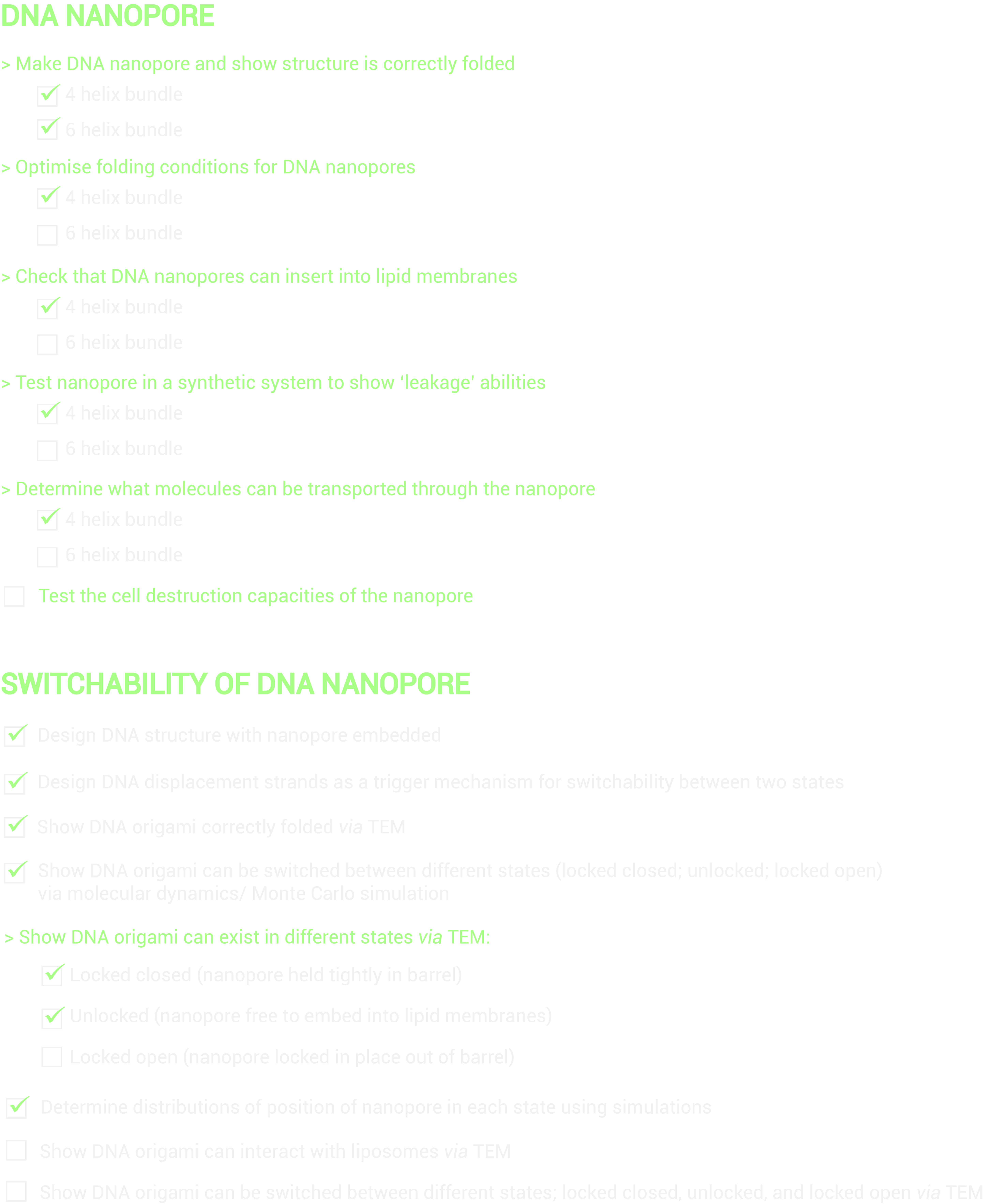
Feasibility
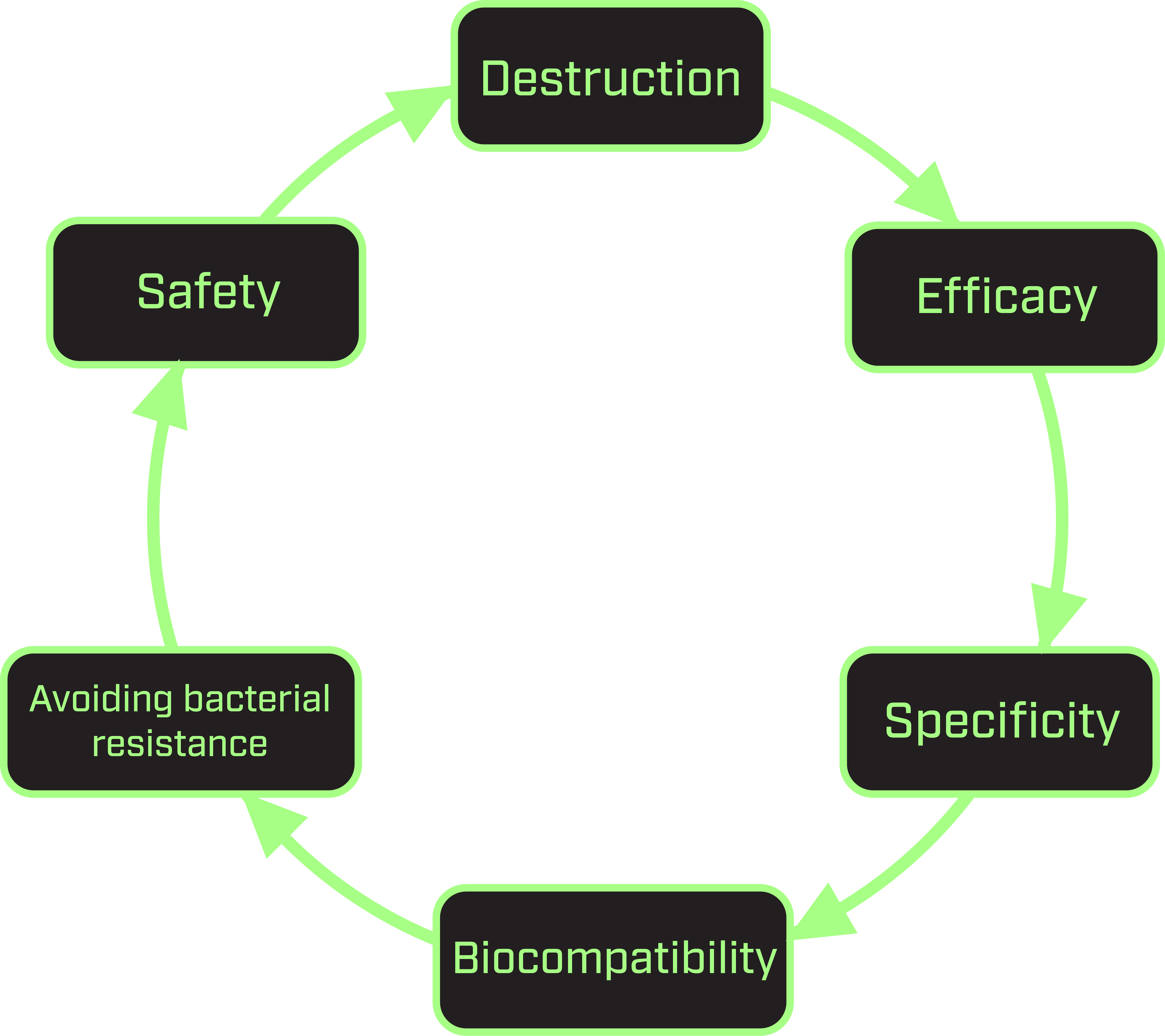
Feasibility of our approach:
To assess the feasibility of this project, we considered the destruction ability, efficacy, specificity, biocompatibility and safety of our DNAlien. This process is critical in determining the potential commercialisation of our product in the medical market as a novel therapeutic.
As our goal was to combat antimicrobial resistance, we aimed to develop a DNA nanopore that could poke holes into microbial membranes induce cell lysis. This is in radically different to the usual method administering small-molecule antibiotic drugs to kill bacteria. The pore consists of DNA helices decorated with cholesterol moieties to allow it to insert into the microbial membrane. Cholesterol plays a critical role in the fluid regulation of bacterial membranes. This dependence lead to the suggestion that similar cholesterol molecules could mediate the insertion of a destruction device. This avoids antimicrobial resistance as the bacterial membrane functionality relies heavily on cholesterol and thus it would not be able to adapt against the device.
Regarding efficacy of bacterial destruction, the concept of poking holes in membranes with pores arose from an established design developed for mammalian cells [5], which induces the host cell to undergo lysis. To begin constructing and designing our nanopore, we recreated this existing pore [5] to validate functionality and demonstrate pore permeability to which we then optimised the conditions to suit our project.
Aptamers were considered and included in our final design of the DNAlien, as there are a number of whole-bacteria targeting aptamers published in the literature [11-13]. To achieve specificity, bacterial targets with known aptamers can be tested for binding of the switchable DNA nanopore to the microbe. With an initial focus on non-pathogenic strains of Escherichia coli, such experiments could be done in a regular biosafety level 1 laboratory setting. To further enhance binding capacity and achieve greater selectivity of targeting specific microbes, additional bacterial markers could be investigated to create a cocktail of aptamers.
In our final DNAlien design, biocompatibility is also considered as the design has to be at least stable enough to reach the bacteria or it would be useless, and off-target effects could lead to detrimental consequences. The former problem could be approached using chemical methods to protect the structure, such as oligolysine [17], and the latter could be tested with mammalian cells. However, the design we have chosen intrinsically guards against off-target effects, by hiding the active pore in an inactive state.
Ultimately, safety also has to be considered as our device would be of little use as a therapeutic if harm was inflicted. Generally, the literature on how the human body reacts to DNA nanostructures in terms of both immune response and therapeutic effects is growing, and our work will benefit from and add to this [18,19]. This will be crucial when undertaking the future design of aptamers to enhance selective targeting, and introducing the DNAlien in vivo.
Feasibility of this project for BIOMOD:
To assess the feasibility of this project for BIOMOD, we referred to the triple constraint in project management which considers the three most significant restrictions in any project; scope, cost and time. We narrowed our project to focus on the destruction of microbes because of the limited timeframe of the competition with the project needing to be delivered within a year. As a result, we focused on the nanopore design and switchability, leaving targeting for future research. In terms of our supervisors’ expertise we decided to work on DNA origami. DNA is also relatively cheap to order, can be delivered in a timely fashion and is easy to work with because of its stability and low toxicity. The lab we were working in was also set up for DNA origami experiments, highlighting the feasibility of this technique for our team in terms of cost and time.
Another aspect of feasibility we considered when defining the scope of the project for BIOMOD was the nature of the project and how linear the computational and experimental steps were. We chose a project that could be broken into many parts and was not sequential, that is the success of one aspect is not dependent of the success of the next. By choosing a non-sequential project many parts of the project could be worked on at once to maximise time efficiency and also to ensure if one aspect of the project fails the whole project would not also fail.
Future
In future work, our short-term goal is to complete our ‘Criteria for success’ checklist (above) and develop a strategy to selectively target specific bacteria, the next stage of the project. Overall, we aim to advance the switching mechanism, improve the ability of destruction by investigating increasing pore size/refining the shape of the pore, maximise transport efficiency as well as conjugate established aptamers to the DNAlien.
Additionally, long-term goals to be considered include developing a solution to target other components of the bacterial cell wall such as the peptidoglycan layer, as well as conduct in vitro testing of the DNAlien on specific strains of bacterial cells. Ultimately, we aim to demonstrate the potential application of our nanodevice as a therapeutic to combat antimicrobial resistance on the medical market.
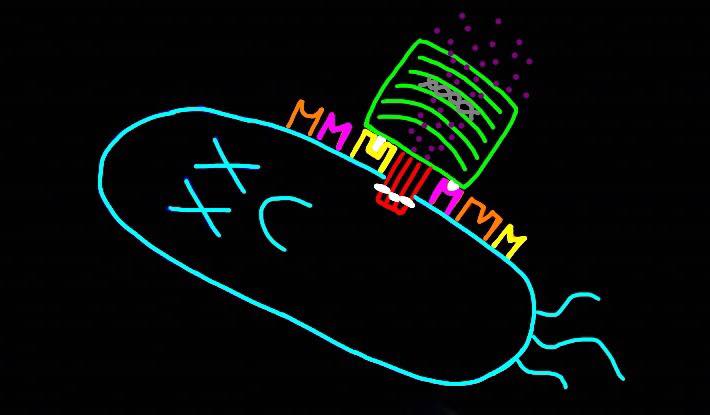
References
[1] Rothemund, P. W. K. Folding DNA to create nanoscale shapes and patterns. Nature 2006, 440, 297.
[2] Benson, E.; Mohammed, A.; Gardell, J.; Masich, S.; Czeizler, E.; Orponen, P.; Hogberg, B. DNA rendering of polyhedral meshes at the nanoscale. Nature 2015, 523, 441-444.
[3] Langecker, M., Arnaut, V., Martin, T.G., List, J., Renner, S., Mayer, M., Dietz, H., and Simmel, F.C. Synthetic Lipid Membrane Channels Formed by Designed DNA Nanostructures. Science 2012, 338, 932–936.
[4] Göpfrich, K.; Zettl, T.; Meijering, A. E. C.; Hernández-Ainsa, S.; Kocabey, S.; Liedl, T.; Keyser, U. F. DNA-Tile Structures Induce Ionic Currents through Lipid Membranes. Nano Lett. 2015, 15 (5), 3134-3138.
[5] Ohmann, A.; Li, C.-Y.; Maffeo, C.; Al Nahas, K.; Baumann, K. N.; Göpfrich, K.; Yoo, J.; Keyser, U. F.; Aksimentiev, A. A synthetic enzyme built from DNA flips 107 lipids per second in biological membranes. Nat. Commun. 2018, 9 (1), 2426.
[6] Burns, J. R.; Göpfrich, K.; Wood, J. W.; Thacker, V. V.; Stulz, E.; Keyser, U. F.; Howorka, S. Lipid-Bilayer-Spanning DNA Nanopores with a Bifunctional Porphyrin Anchor. Angew. Chem. Int. Ed. 2013, 52 (46), 12069-12072.
[7] Review on Antimicrobial Resistance. https://amr-review.org/ [accessed 4 Oct. 2018].
[8] Walsh, F. BBC. https://www.bbc.com/news/health-30416844 [accessed 4 Oct. 2018].
[9] Zhang, L.-J.; Gallo, R. L. Antimicrobial peptides. Curr. Biol. 2016, 26 (1), R14-R19.
[10] Sato, H.; Feix, J. B. Peptide–membrane interactions and mechanisms of membrane destruction by amphipathic α-helical antimicrobial peptides. Biochim. Biophys. Acta Biomembr. 2006, 1758 (9), 1245-1256.
[11] Kim, Y.S., Song, M.Y., Jurng, J., and Kim, B.C. (2013). Isolation and characterization of DNA aptamers against Escherichia coli using a bacterial cell–systematic evolution of ligands by exponential enrichment approach. Analytical Biochemistry 2013 436, 22–28.
[12] Cao, X., Li, S., Chen, L., Ding, H., Xu, H., Huang, Y., Li, J., Liu, N., Cao, W., Zhu, Y., et al. Combining use of a panel of ssDNA aptamers in the detection of Staphylococcus aureus. Nucleic Acids Res 2009 37, 4621–4628.
[13] Chen, F., Zhou, J., Luo, F., Mohammed, A.-B., and Zhang, X.-L. Aptamer from whole-bacterium SELEX as new therapeutic reagent against virulent Mycobacterium tuberculosis. Biochemical and Biophysical Research Communications 2007 357, 743–748.
[14] Kim, Y. S.; Song, M. Y.; Jurng, J.; Kim, B. C. Isolation and characterization of DNA aptamers against Escherichia coli using a bacterial cell–systematic evolution of ligands by exponential enrichment approach. Analytical Biochemistry 2013 436, 22–28.
[15] Torres, A. G.; Li, Y.; Tutt, C.B.; Xin, L.; Eaves-Pyles, T.; Soong, L. Outer Membrane Protein A of Escherichia coli O157:H7 Stimulates Dendritic Cell Activation. Infect. Immune. 2006, 74 (5), 2676–2685.
[16] Ghosh, N.; McKillop, T.J.; Jowitt, T.A,; Howard, M.; Davies, H.; Holmes, D.F.; Roberts, I.S.; Bella, J. Collagen-Like Proteins in Pathogenic E. coli Strains. PLoS One. 2012, 7 (6), e37872.
[17] Ponnuswamy, N.; Bastings, M. M. C.; Nathwani, B.; Ryu J. H.; Chou, L.Y.T.; Vinther, M.; Aileen Li, W.; Anastassacos, F. M.; Mooney, D. J; Shih, W.M. Oligolysine-based coating protects DNA nanostructures from low-salt denaturation and nuclease degradation Nat. Commun. 2017, 8, 15654.
[18] Li, S., Jiang, Q., Liu, S., Zhang, Y., Tian, Y., Song, C., Wang, J., Zou, Y., Anderson, G.J., Han, J.-Y., et al. A DNA nanorobot functions as a cancer therapeutic in response to a molecular trigger in vivo. Nature Biotechnology 2018.
[19] Perrault, S.D., and Shih, W.M. Virus-Inspired Membrane Encapsulation of DNA Nanostructures To Achieve In Vivo Stability. ACS Nano 2014 8, 5132–5140.